H2 promotes autophagy to reduce LDL-induced inflammationScientific Research
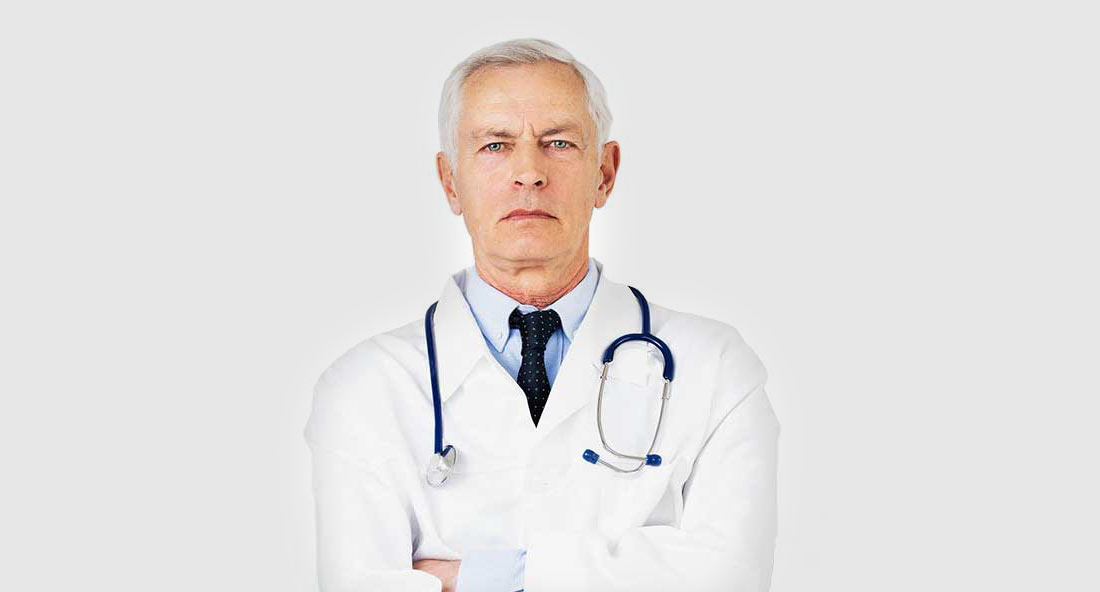
Hydrogen attenuated oxidized low-density lipoprotein-induced inflammation through the stimulation of autophagy via sirtuin 1
Sen Yang
Department of Vascular Surgery, Tianjin First Central Hospital, Tianjin 300192, P.R. China
Ju He
Department of Vascular Surgery, Tianjin First Central Hospital, Tianjin 300192, P.R. China
Xiaofeng Li
Department of Vascular Surgery, Tianjin First Central Hospital, Tianjin 300192, P.R. China
Hui Liu
Department of Vascular Surgery, Tianjin First Central Hospital, Tianjin 300192, P.R. China
Jian Zhao
Department of Vascular Surgery, Tianjin First Central Hospital, Tianjin 300192, P.R. China
Mingming Liu
Department of Vascular Surgery, Tianjin First Central Hospital, Tianjin 300192, P.R. China
Associated Data
- Data Availability Statement
The datasets used and/or analyzed during the current study are available from the corresponding author on reasonable request.
Abstract
Chronic inflammation is a central pathogenic mechanism underlying the induction and progression of atherosclerosis (AS). Hydrogen has been demonstrated to serve a protective role in diverse models of disease. However, the potential effects and mechanism of hydrogen with respect to ox-LDL-induced inflammation have not yet been completely elucidated. In the present study, various concentrations (0, 50 and 100 mg/l) of oxidized low-density lipoprotein (ox-LDL) were used to treat RAW264.7 cells. A Cell Counting kit-8 assay was used to determine cell viability and western blot analysis was performed to determine the expression of proteins that are involved in autophagy. The expression of inflammatory cytokines in ox-LDL-treated macrophages was detected using ELISA. Small interfering (si)RNA against sirtuin 1 (SIRT1) was employed to investigate the mechanism underlying hydrogen-activated autophagy. The results indicated that ox-LDL stimulation promoted inflammatory cytokine expression and impaired autophagic flux in RAW264.7 cells. Furthermore, hydrogen inhibited ox-LDL-induced inflammatory cytokine expression by upregulating autophagic flux. SIRT1 mediated the upregulation of autophagic flux via hydrogen in ox-LDL-treated macrophages. To conclude, the present study provided novel insights into the role of defective autophagy in the pathogenesis of AS and identified autophagy to be a promising therapeutic target for the treatment of AS. Notably, hydrogen may represent a potential agent for the treatment of AS.
Introduction
Atherosclerosis (AS), a chronic inflammatory disorder, is the most prevalent cardiovascular disease and the world’s leading cause of fatality and morbidity (1–3). Evidence has indicated that modified lipids, particularly oxidized low-density lipoprotein (ox-LDL), are predominantly engulfed by macrophages, resulting in increased pro-inflammatory cytokine expression and further macrophage recruitment, thus contributing to increases in the size and complexity of atherosclerotic plaques (4–7). Therefore, it is of crucial importance to investigate the interaction between macrophages and modified lipids as well as the regulatory mechanisms of inflammation in macrophages.
Autophagy, a highly controlled intracellular process whereby long-lived proteins and damaged organelles are degraded and recycled, has an essential role in cell survival and in responses to environmental or internal pressure (8–12). Recent studies have demonstrated that autophagy is involved in complicated interactions with the innate and adaptive immunity, and thus is associated with the pathogenesis of inflammatory diseases (13–17). Macrophages lacking autophagy-related protein 16-1 (Atg16L1) or autophagy-related (Atg)7, key components of the autophagic process, resulted in upregulated secretion of interleukin (IL)-1β and IL-18 in response to inflammatory stimulation (13). Using mice with knocked out autophagy protein Atg5 in macrophages and neutrophils, researchers identified that Atg5 serves an important role in resistance to the intracellular pathogens Listeria monocytogenes and Toxoplasma gondii (18). Atg5 was required for interferon (IFN)-γ/lipopolysaccharide (LPS)-induced harm to the T. gondii parasitophorous vacuole membrane, and thus the elimination of intracellular pathogens (18). With respect to the pathogenesis of AS, studies have reported that ox-LDL-induced autophagy impairment in HUVECs contributes to the development of this disease (19). Furthermore, autophagy dysfunction in macrophages may accelerate atherosclerotic progression in vivo (20). Thus, modulation of autophagy may lead to therapeutic interventions for AS.
Hydrogen, possessing anti-oxidative properties by selectively neutralizing cytotoxic reactive oxygen species, exerts organ-protective effects by regulating oxidative stress, inflammation and apoptosis (21). Recently, accumulating evidence has demonstrated the protective role of hydrogen on diverse models of disease, including diseases of the central nervous system, cardiovascular system, lungs and renal system (21–26). Hydrogen treatment attenuated LPS-induced upregulation of pro-inflammatory cytokines, including tumor necrosis factor (TNF)-α, IL-1β and high mobility group box 1 in macrophages (27). Chronic hydrogen-rich saline treatment depressed pro-inflammatory cytokines expression, including IL-6 and IL-1β (28). In addition, hydrogen-rich saline mediates neuroprotection and attenuates acute kidney injury through the regulation of autophagy (29,30). Oral treatment with hydrogen-rich water for 6 months alleviated the progression of AS in apolipoprotein E-knockout mice (31). However, the therapeutic mechanism underlying its protective effects has not yet been completely elucidated. Considering the inhibited effect of autophagy on inflammation and the promoted effect of hydrogen on autophagy (32,33), the present study intended to explore the effect of hydrogen on ox-LDL-induced inflammation and to investigate the potential role of autophagy in hydrogen-mediated protection.
Materials and methods
Reagents
Bovine serum albumin (BSA; cat. no. A4161), rapamycin (cat. no. R117), bafilomycin A1 (BafA1; cat. no. B1793) and protease inhibitor cocktail (cat. no. P8340) were purchased from Sigma-Aldrich (Merck KGaA, Darmstadt, Germany). Ox-LDL was obtained from Yiyuan Biotech Co., Ltd (Guangzhou, Guangdong, China). RPMI-1640 medium and fetal bovine serum were obtained from Gibco (Thermo Fisher Scientific, Inc., Waltham, MA, USA). Radioimmunoprecipitation assay (RIPA) lysis buffer (cat. no. P0013B) was purchased from Beyotime Institute of Biotechnology (Haimen, China).
Hydrogen preparation
As previously described (21,27), purified hydrogen was dissolved into RPMI-1640 medium at 0.4 MPa pressure for 2 h to reach saturation. O2 was dissolved into a second medium by bubbling O2 gas at the saturated level (42.5 mg/l), and CO2 into a third medium by bubbling CO2 gas. A total of 0.6 mM hydrogen medium was prepared by combining the three media (H2 medium:O2 medium:CO2 medium) in the proportion of 90%:5%:5% (v/v/v). The control medium was prepared by combining two media (95% O2 medium:5% CO2 medium). H2, O2 and CO2 concentrations were confirmed with gas chromatography.
Cell culture
The mouse macrophage-like cell line Raw264.7, obtained from the Type Culture Collection of the Chinese Academy of Sciences (Shanghai, China), was cultured in RPMI-1640 medium supplemented with 10% fetal bovine serum and maintained at 37°C in an atmosphere containing 5% CO2.
Cell viability assay
Cells were seeded in 96-well plates at a density of 1×106 cells/well and were cultured with or without various concentrations (0.00, 0.15, 0.30 and 0.60 mM) of hydrogen for 24 h at 37°C. Subsequently, cell viability was determined using water-soluble tetrazolium salt-8 staining with a CCK8 (Cell Counting kit-8) assay, (cat. no. CK04; Dojindo Molecular Technologies, Inc., Kumamoto, Japan) according to the manufacturer’s instructions. Optical density was determined at 450 nm with a plate reader (Multiskan FC; Thermo Fisher Scientific, Inc.).
Cell transfection experiment
Control small interfering (si)RNA (non-targeting; siControl) and siRNA specific for mouse sirtuin 1 (SIRT1; ON-TARGETplus SMARTpool; cat. no. L-303540-00) were obtained from GE Healthcare Dharmacon, Inc. (Lafayette, CO, USA). The sequences of the SIRT1 siRNAs utilized are presented in Table I. A total of 20 nM siRNA was transfected into osteoblasts using Lipofectamine 2000 (cat. no. 11668027; Invitrogen; Thermo Fisher Scientific, Inc.), as reported previously (34). Cells were incubated for 24 h before being used for subsequent experiments.
Table I.
Sequences of SIRT1 siRNAs.
SIRT1 siRNAs | Sequence |
---|---|
1 SIRT1 siRNA sense | 5′-GAUGAAGUUGACCUCCUCATT-3′ |
1 SIRT1 siRNA antisense | 5′-UGAGGAGGUCAACUUCAUCTT-3′ |
2 SIRT1 siRNA sense | 5′-TCAGTGTCATGGTTCCTTTGC-3′ |
2 SIRT1 siRNA antisense | 5′-AATCTGCTCCTTTGCCACTCT-3′ |
3 SIRT1 siRNA sense | 5′-GCAACAGCAUCUUGCCUGACUUGUA-3′ |
3 SIRT1 siRNA antisense | 5′-UCAUAGAGCCAUGAAGUAUGACAAA-3′ |
4 SIRT1 siRNA sense | 5′-TTTCAGAACCACCAAAGCG-3′ |
4 SIRT1 siRNA antisense | 5′-TCCCACAGGAAACAGAAACC-3′ |
siRNA, small interfering RNA; SIRT1, sirtuin 1.
Western blot analysis
Cells were lysed in RIPA lysis buffer with a protein inhibitor cocktail for 30 min on ice, and the lysates were centrifuged at 12,000 × g for 10 min at 4°C. The supernatants were collected, and 12 or 15% SDS-PAGE was used to separate the proteins before transfer to polyvinylidene fluoride membranes (EMD Millipore; Billerica, MA, USA). The membranes were blocked with 5% non-fat milk for 1 h at room temperature, followed by incubation with the following primary antibodies: Anti-microtubule-associated protein 1A/1B-light chain 3 (LC3; 1:1,000; cat. no. L7543; Sigma-Aldrich; Merck KgaA), anti-p62 (1:1,000; cat. no. 39749), anti-SIRT1 (1:1,000; cat. no. 8469; both Cell Signaling Technology, Inc., Danvers, MA, USA) and anti-GAPDH (1:1,000; cat no. A01020; Abbkine Scientific Co., Ltd., Wuhan, China) at 4°C overnight. Membranes were washed with Tris-buffered saline with Tween-20 (20 mM Tris-HCl buffer; pH=7.4; containing 150 mM NaCl and 0.05% Tween-20) three times and then incubated with horseradish peroxidase (HRP)-conjugated anti-rabbit immunoglobulin G (1:2,000; cat. no. 7074; Cell Signaling Technology, Inc.) and HRP-conjugated anti-mouse IgG antibodies (1:1,000; cat. no. sc-2005; Santa Cruz Biotechnology, Inc., Dallas, TX, USA) at room temperature for 2 h. After probing with specific primary antibodies and an HRP-conjugated secondary antibody, the protein bands were detected using a super ECL plus detection reagent (Thermo Fisher Scientific, Inc.), and the band density was analyzed using ImageJ 1.41 (National Institutes of Health; Bethesda, MD, USA).
ELISA
The levels of inflammatory cytokines [IL-6 (cat. no. CME0006), TNF-α (cat. no. CME0004) and IL-1β (cat. no. CME0015)] were detected using ELISA kits (4A Biotech Co. Ltd., Beijing China). All procedures were performed in accordance with the manufacturer’s instructions.
Statistical analysis
Results were expressed as the mean ± standard error of the mean. The differences between groups were analyzed using the Brown-Forsythe test and, if appropriate, by one-way analysis of variance followed by Dunnett’s post hoc test or the Bonferroni test. P<0.05 was considered to indicate a statistically significant difference.
Results
Ox-LDL impairs autophagic flux in macrophages
In the present study, western blotting of LC3-II/GAPDH ratios in ox-LDL-treated RAW264.7 cells was performed. As indicated in Fig. 1A and B, the expression level of LC3-II was significantly increased in macrophages treated with 100 mg/l ox-LDL compared with that of the control. The increased level of LC3-II could be due to increased initiation of autophagy or decreased clearance of autophagosomes, as reflected by accumulation of the autophagic substrate p62. Therefore, the protein expression levels of p62 in ox-LDL co-cultured macrophages were measured. When compared with the control, ox-LDL significantly increased p62 expression levels in macrophages (Fig. 1A and C). To further interpret the decreased clearance of autophagosomes, BafA1, which inhibits the late degradation stage of autophagy. Results indicated that ox-LDL significantly elevated the expression levels of LC3-II and p62 relative to the control (Fig. 1D-F). However, the ox-LDL-induced increase in LC3-II and p62 was nullified in the presence of BafA1 (Fig. 1D-F). These results demonstrated that ox-LDL impaired autophagic flux in macrophages.
Ox-LDL impaired autophagic flux in RAW264.7 cells. (A) Western blots performed after RAW264.7 cells were treated with various concentrations (0, 50, 100 mg/l) of ox-LDL for 24 h. (B and C) The density of the western blot bands indicated in (A) was quantified using ImageJ software. (D) Western blots performed once RAW264.7 cells were incubated with/without bafilomycin A1 (50 nM), prior to being stimulated with/without ox-LDL (100 mg/l) for 24 h. (E and F) The density of the western blot bands indicated in (D) was quantified using ImageJ software. The data are presented as the mean ± standard error of the mean from three independent experiments. *P<0.05 as indicated. N.S, not significant. BafA1, bafilomycin A1; LC3, microtubule-associated protein 1A/1B-light chain 3; Ox-LDL, oxidized low-density lipoprotein.
Ox-LDL-induced inflammation in macrophages
To explore the effects of ox-LDL on inflammatory cytokine expression in macrophages, RAW264.7 cells were exposed to various concentrations of ox-LDL. The results indicated that ox-LDL dose-dependently increased the expression of cytokines (Fig. 2). At a concentration of 100 mg/l, the levels of TNF-α were increased by almost 7-fold; those of IL-6 were increased by 2-fold; and those of IL-1β were increased by 4-fold (Fig. 2).
Ox-LDL induced inflammatory cytokine expression in RAW264.7 cells. The expression of inflammatory cytokines, (A) TNF-α, (B) IL-6 and (C) IL-1β, in RAW264.7 cells following incubation with various concentrations (0, 50 and 100 mg/l) of ox-LDL for 24 h. The cytokine concentrations were quantified by ELISA. Data are presented as the mean ± standard error of the mean from three independent experiments. *P<0.05 as indicated. TNF-α, tumor necrosis factor-α; IL, interleukin; Ox-LDL, oxidized low-density lipoprotein.
Hydrogen enhances autophagic flux in macrophages
Previous studies have suggested that hydrogen triggers autophagy in several diseases (29,30). In the present study, it was investigated whether hydrogen could activate autophagy in macrophages. Firstly, the results indicated that hydrogen did not impair the cell viability at the selected dose (Fig. 3A). Following this, the effect of hydrogen on autophagic flux was assessed. Notably, hydrogen increased the protein expression levels of LC3-II in a dose-dependent manner (Fig. 3B and C). Furthermore, 0.30 and 0.60 mM hydrogen significantly reduced the expression level of p62 in macrophages (Fig. 3B and D). These results indicated that treatment with hydrogen enhanced autophagic flux in macrophages.
Activation of autophagic flux following exposure to hydrogen in RAW264.7 cells. (A) Cell Counting kit-8 assay of RAW264.7 cells treated with various concentrations (0.00, 0.15, 0.30 and 0.60 mM) of hydrogen for 24 h. (B) Western blots performed after RAW264.7 cells were treated with various concentrations (0.00, 0.15, 0.30 and 0.60 mM) of hydrogen for 24 h. The density of the western blot bands for (C) LC3-II and (D) p62 indicated in (B) was quantified using ImageJ software. The data are presented as the mean ± standard error of the mean from three independent experiments. *P<0.05 as indicated. N.S, not significant; LC3, microtubule-associated protein 1A/1B-light chain 3.
Enhanced autophagic flux mediates the anti-inflammatory effect of hydrogen in ox-LDL-treated macrophages
The above results demonstrated that ox-LDL increased inflammatory cytokine expression levels and impaired autophagic flux in macrophages. In Fig. 4A-C, the data suggest that hydrogen downregulated cytokines in a dose-dependent manner in macrophages. To further investigate whether the effect of hydrogen on ox-LDL-induced cytokines was involved in autophagic flux, RAW264.7 cells were pretreated with BafA1 (an inhibitor of autophagy) or rapamycin (an activator of autophagy) before being cultured with ox-LDL or ox-LDL and hydrogen. As indicated in Fig. 4D-F, rapamycin significantly decreased the expression of cytokines induced by ox-LDL. Similarly, the expression of cytokines induced by ox-LDL was lower in hydrogen cotreated cells compared with the ox-LDL alone group. In addition, inhibition of hydrogen-induced autophagy by BafA1 partly reversed its effects on cytokine expression. Collectively, these results indicated that hydrogen ameliorated ox-LDL-induced inflammation, and the anti-inflammatory effect was mediated by enhanced autophagic flux.
Hydrogen inhibited ox-LDL-induced inflammatory cytokine expression by upregulation of autophagic flux in RAW264.7 cells. The expression of (A) TNF-α, (B) IL-6 and (C) IL-1β after RAW264.7 cells were incubated with/without hydrogen (0.00, 0.15, 0.30 and 0.60 mM), prior to being stimulated with/without ox-LDL (100 mg/l) for 24 h. The expression of (D) TNF-α, (E) IL-6 and (F) IL-1β after RAW264.7 cells were incubated with/without hydrogen (0.60 mM), bafilomycin A1 (50 nM) and/or rapamycin (200 nM), prior to being stimulated with/without ox-LDL (100 mg/l) for 24 h. The cytokine concentrations were quantified by ELISA. Data are presented as the mean ± standard error of the mean from three independent experiments. *P<0.05 as indicated. IL, interleukin; BafA1, bafilomycin A1; Rap, rapamycin; TNF-α, tumor necrosis factor-α; Ox-LDL, oxidized low-density lipoprotein.
Upregulation of SIRT1 is essential for the rescuing effect of hydrogen on autophagy flux in ox-LDL-treated macrophages
SIRT1, a member of the NAD+-dependent deacetylases, serves an important role in regulating autophagic flux (35,36). Recently, studies have demonstrated that SIRT1 is involved in the inflammatory responses of various conditions (37). In the present study, the expression of the SIRT1 protein in ox-LDL-stimulated macrophages was investigated (Fig. 5). Ox-LDL treatment significantly decreased the expression of SIRT1 protein in a dose-dependent manner (Fig. 5A and B). However, co-treatment with hydrogen significantly rescued the expression level of SIRT1 protein in ox-LDL-stimulated macrophages (Fig. 5C and D). To investigate whether the ability of hydrogen to ameliorate impaired autophagic flux depends on SIRT1, siRNA was used to knock down SIRT1 (Fig. 5E-G and J). As indicated in Fig. 5G and H, following the transfection with SIRT1 siRNA, the expression level of LC3-II was significantly increased. Furthermore, the downregulating effect of hydrogen on p62 expression was abolished (Fig. 5G and I). These results indicated that SIRT1 is essential for the ameliorating the effect of hydrogen on ox-LDL-induced impaired autophagic flux in macrophages.
SIRT1 mediated the upregulation of autophagic flux by hydrogen in ox-LDL-treated RAW264.7 cells. (A) Western blots performed after RAW264.7 cells were treated with various concentrations (0, 50 and 100 mg/l) of ox-LDL for 24 h. (B) The density of the western blot bands indicated in (A) was quantified using ImageJ software. (C) Western blots performed after RAW264.7 cells were incubated with/without hydrogen (0.60 mM) prior to being stimulated with/without ox-LDL (100 mg/l) for 24 h. (D) The density of the western blot bands indicated in (C) was quantified using ImageJ software. (E) Western blots performed after RAW264.7 cells were treated with siControl or siSIRT1 for 24 h. (F) The density of the western blot bands indicated in (E) was quantified using ImageJ software. (G) Western blots performed after RAW264.7 cells were cultured in siControl or siSIRT1, prior to treatment with ox-LDL (100 mg/l) and/or hydrogen (0.60 mM) for 24 h. The density of the western blot bands for (H) LC3-II, (I) p62 and (J) SIRT1 indicated in (G) was quantified using ImageJ software. The data are presented as the mean ± standard error of the mean from three independent experiments. *P<0.05 as indicated. N.S, not significant; LC3, microtubule-associated protein 1A/1B-light chain 3; SIRT1, sirtuin 1; Ox-LDL, oxidized low-density lipoprotein; si, small interfering.
Discussion
AS predisposes patients to various cardiovascular diseases, including hypertension, coronary heart disease and stroke (38–41). Ox-LDL-induced inflammation serves a crucial role in the pathogenesis of atherosclerosis. Hydrogen, as a novel antioxidant, can exert potent cellular protective effects in different disease models (22). Notably, oral administration of hydrogen-rich water for 6 months prevented the progress of atherosclerosis in an animal model (31). Furthermore, hydrogen represents a novel therapeutic choice for cardiovascular disease (22). However, the molecular mechanisms require elucidation. In the present study, the present data suggested that hydrogen attenuated ox-LDL-induced inflammatory cytokine expression through the stimulation of autophagy via SIRT1.
Hydrogen, as the lightest and most abundant chemical element, may act as a scavenger to selectively ameliorate reactive oxygen species (ROS) and exert potent cellular protective effects in a variety of clinical and experimental models of diseases (21,30). In rats, hydrogen gas reduced infarct sizes without affecting hemodynamic parameters during ischemia-reperfusion (I/R) injury of the left anterior descending coronary artery (42). Intraperitoneal injection of hydrogen-rich saline exerted cardioprotection against I/R injury by reducing plasma and myocardial malondialdehyde concentrations, cardiac cell apoptosis and caspase-3 activity (43). Furthermore, continued consumption of hydrogen water decreased the oxidative stress level in the aorta and reduced the number of atherosclerotic lesions in a previous study (31). In the present study, the effects of hydrogen on ox-LDL-treated macrophages were examined in vitro, and the results indicated that hydrogen markedly decreased the secretion of pro-inflammatory cytokines compared with the control. Considering the pivotal role of ox-LDL-induced inflammation in the pathogenesis of AS, hydrogen may serve as an anti-inflammatory agent and have therapeutic potential in the treatment of atherosclerosis.
Autophagy is a key component of the cellular stress adaptation responses that maintain cellular homeostasis. It is important in protection against different types of disease, including neurodegenerative disease, aging, cancer and inflammatory illness (44–46). Perturbations in autophagy contribute to chronic inflammatory diseases and autoimmune diseases, including Crohn’s disease, systemic lupus erythematosus, obesity and diabetes (47). In mice, loss of autophagy via knockout of Atg5 in macrophages results in enhanced systemic and hepatic inflammation, which is due to the disturbance between pro-inflammatory M1 and anti-inflammatory M2 polarization (48). Atg16L1-deficient activated caspase-1 by Toll/IL-1 receptor domain-containing adaptor inducing IFN-β in macrophages and resulted in increased secretion of the inflammatory cytokines IL-1β and IL-18 (49). The present data revealed that ox-LDL treatment resulted in impaired autophagic flux. Furthermore, enhanced autophagy by rapamycin (a classical autophagy activator) or hydrogen decreased the effects on ox-LDL-induced pro-inflammatory cytokine expression. However, downregulation of autophagy by bafilomycin A1 alleviated the inhibitory effect of hydrogen on pro-inflammatory cytokine secretion.
SIRT1, belonging to the silent information regulator 2 family, has been reported to be involved in age-associated diseases, including neurodegenerative diseases, metabolic diseases, cardiovascular diseases and osteoporosis (50). The target proteins of SIRT1 include those associated with regulating cell proliferation, DNA damage repair and gene transcription (51). A recent study suggested that SIRT1 regulates autophagy through several cellular pathways, either directly or indirectly (35). Previous results have indicated that activation of SIRT1 can induce autophagy, whereas SIRT1-deficient mouse embryonic fibroblasts insufficiently activated autophagy under starvation conditions (35,52). In hepatocellular carcinoma cells, inhibition of SIRT1 was demonstrated to impair cell proliferation and rapamycin-induced autophagy (35). In addition, overexpression of SIRT1 in neurons prevents the accumulation of the prion protein and neurotoxicity by inducing autophagy (52). In human colorectal or cervical cancer cells, SIRT1 is necessary for the induction of autophagy under nutrient deprivation or caloric restriction conditions, whereas knockdown of SIRT1 expression hampers the activation of autophagy (53). The present data suggested that SIRT1 was involved in alleviating the impairment of autophagy caused by hydrogen. With siRNAs against SIRT1, the hydrogen-trigged enhancing effect on autophagy was abolished in ox-LDL-treated macrophages. In conclusion, the present study demonstrated that ox-LDL decreased the expression of SIRT1, resulting in impaired autophagic flux, which consequently resulted in the upregulation of pro-inflammatory cytokines. Furthermore, the findings suggested that hydrogen may enhance autophagy via increasing SIRT1 expression and decreasing ox-LDL-induced inflammation. The present study provided novel insights into the role of defective autophagy in the pathogenesis of AS and identified autophagy as a promising therapeutic target for the treatment of AS. Furthermore, hydrogen may represent a potential agent for treating AS.
Funding
The present study was supported by Tianjin Municipal Health Bureau of Science and Technology Fund Projects (grant no. 2014KZ024).
Availability of data and materials
The datasets used and/or analyzed during the current study are available from the corresponding author on reasonable request.
Authors’ contributions
The current study was designed by JH and SY. SY, XL and HL performed western blotting, ELISA and CCK8 assays. SY, XL and JZ helped with hydrogen preparation and cell culture. The statistical analysis was conducted by ML. All authors read and approved the final version of the manuscript.
References
The Original Article:
original title: Hydrogen attenuated oxidized low‑density lipoprotein‑induced inflammation through the stimulation of autophagy via sirtuin 1
Sen Yang, Hu He, Xiaofeng Li, Hui Liu, Jian Zhao, Mingming Liu
-
Abstract:
Chronic inflammation is a central pathogenic mechanism underlying the induction and progression of atherosclerosis (AS). Hydrogen has been demonstrated to serve a protective role in diverse models of disease. However, the potential effects and mechanism of hydrogen with respect to ox-LDL-induced inflammation have not yet been completely elucidated. In the present study, various concentrations (0, 50 and 100 mg/l) of oxidized low-density lipoprotein (ox-LDL) were used to treat RAW264.7 cells. A Cell Counting kit-8 assay was used to determine cell viability and western blot analysis was performed to determine the expression of proteins that are involved in autophagy. The expression of inflammatory cytokines in ox-LDL-treated macrophages was detected using ELISA. Small interfering (si)RNA against sirtuin 1 (SIRT1) was employed to investigate the mechanism underlying hydrogen-activated autophagy. The results indicated that ox-LDL stimulation promoted inflammatory cytokine expression and impaired autophagic flux in RAW264.7 cells. Furthermore, hydrogen inhibited ox-LDL-induced inflammatory cytokine expression by upregulating autophagic flux. SIRT1 mediated the upregulation of autophagic flux via hydrogen in ox-LDL-treated macrophages. To conclude, the present study provided novel insights into the role of defective autophagy in the pathogenesis of AS and identified autophagy to be a promising therapeutic target for the treatment of AS. Notably, hydrogen may represent a potential agent for the treatment of AS.